GENE
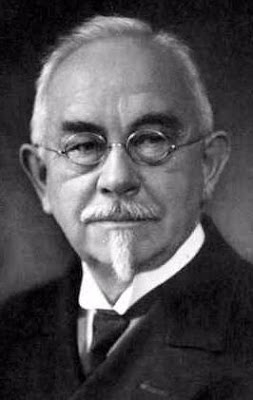 |
WILHELM JOHANSEN |
This article is about the heritable unit for the transmission of biological traits. A gene is a sequence of DNA or RNA that codes for a molecule that has a function.
A chromosome unraveling into a long string of DNA, a section of which is highlighted as the gene
Chromosome(107 - 1010 bp) DNAGene(103 - 106 bp )Function
The image above contains clickable links A
gene is a region of
DNA that encodes function. A
chromosome consists of a long strand of
DNA containing many genes. A human chromosome can have up to 500 million base pairs of
DNA with thousands of genes.
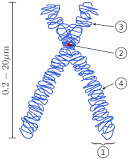 |
Diagram of a replicated and condensed metaphase eukaryotic chromosome.
(1) Chromatid – one of the two identical parts of the chromosome after the S phase. (2) Centromere – the point where the two chromatids touch. (3) Short arm (p). (4) Longarm (q).
Hammond CM, Strømme CB, Huang H, Patel DJ, Groth A (March 2017). "Histone chaperone networks shaping chromatin function". Nature Reviews. Molecular Cell Biology.
Wilson, John (2002). Molecular biology of the cell: a problems approach. New York: Garland Science
|
In biology, a
gene is a sequence of nucleotides in
DNA or
RNA that encodes the synthesis of a
gene product, either
RNA or
protein.
During gene expression, the
DNA is first copied into
RNA. The
RNA can be directly functional or be the intermediate template for a protein that performs a function. The transmission of
genes to an organism's offspring is the basis of the inheritance of the
phenotypic traits. These genes make up different
DNA sequences called
genotypes. Genotypes along with environmental and developmental factors determine what the
phenotypes will be.
Most biological traits are under the influence of polygenes (many different genes) as well as gene-environment interactions. Some genetic traits are instantly visible, such as eye color or the number of limbs, and some are not, such as blood type, the risk for specific diseases, or the thousands of basic biochemical processes that constitute life.
Genes can acquire mutations in their sequence, leading to different variants, known as alleles, in the population. These alleles encode slightly different versions of a protein, which cause different phenotypical traits. Usage of the term "having a gene" (e.g., "good genes," "hair color gene") typically refers to containing a different
allele of the same, shared gene.[1]
Genes evolve due to natural selection/survival of the fittest and genetic drift of the alleles.
The concept of a gene continues to be refined as new phenomena are discovered.[2] For example, regulatory regions of a gene can be far removed from its coding regions, and coding regions can be split into several exons. Some viruses store their genome in RNA instead of DNA and some gene products are functional non-coding RNAs. Therefore, a broad, modern working definition of a gene is any discrete locus of heritable, genomic sequence which affects an organism's traits by being expressed as a functional product or by regulation of gene expression.[3][4]
The term
gene was introduced by Danish botanist, plant physiologist and geneticist
Wilhelm Johannsen in 1909.[5] It is inspired by the ancient Greek:
γόνος, Logos, that means offspring and procreation.
History
Photograph of Gregor Mendel
Gregor Mendel
History of genetics
Discovery of discrete inherited units
The existence of discrete inheritable units was first suggested by Gregor Mendel (1822–1884).[6] From 1857 to 1864, in Brno, Austrian Empire (today's Czech Republic), he studied inheritance patterns in 8000 common edible pea plants, tracking distinct traits from parent to offspring. He described these mathematically as 2n combinations where n is the number of differing characteristics in the original peas. Although he did not use the term gene, he explained his results in terms of discrete inherited units that give rise to observable physical characteristics.
This description prefigured
Wilhelm Johannsen's distinction between genotype (the genetic material of an organism) and phenotype (the observable traits of that organism). Mendel was also the first to demonstrate independent assortment, the distinction between
dominant and recessive traits, the distinction between a
heterozygote and
homozygote, and the phenomenon of
discontinuous inheritance.
Prior to Mendel's work, the dominant theory of heredity was one of blending inheritance, which suggested that each parent contributed fluids to the fertilization process and that the traits of the parents blended and mixed to produce the offspring.
Charles Darwin developed a theory of inheritance he termed
pangenesis, from Greek pan ("all, the whole") and genesis ("birth") /
genos ("origin").[7][8]
Darwin used the term
gemmule to describe hypothetical particles that would mix during reproduction.
Mendel's work went largely unnoticed after its first publication in 1866 but was rediscovered in the late 19th century by Hugo de Vries, Carl Correns, and Erich von Tschermak, who (claimed to have) reached similar conclusions in their own research.[9] Specifically, in 1889, Hugo de Vries published his book Intracellular Pangenesis,[10] in which he postulated that different characters have individual hereditary carriers and that inheritance of specific traits in organisms comes in particles. De Vries called these units "pangenes" (Pangens in German), after Darwin's 1868 pangenesis theory.
Sixteen years later, in 1905, Wilhelm Johannsen introduced the term 'gene'[5] and William Bateson that of 'genetics'[11] while Eduard Strasburger, amongst others, still used the term 'pangene' for the fundamental physical and functional unit of heredity.[10]: Translator's preface, viii
Discovery of DNA
Advances in understanding genes and inheritance continued throughout the 20th century.
Deoxyribonucleic acid (
DNA) was shown to be the molecular repository of genetic information by experiments in th
e 1940s to 1950s.[12][13] The structure of DNA was studied by Rosalind Franklin and
Maurice Wilkins using X-ray crystallography, which led James
D. Watson and
Francis Crick to publish a model of the double-stranded DNA molecule whose paired nucleotide bases indicated a compelling hypothesis for the mechanism of genetic replication.[14][15]
In the early 1950s, the prevailing view was that the genes in a chromosome acted like discrete entities, indivisible by recombination and arranged like beads on a string. The experiments of
Benzer using mutants defective in the rII region of bacteriophage T4 (1955–1959) showed that individual genes have a simple linear structure and are likely to be equivalent to a linear section of
DNA.[16][17]
Collectively, this body of research established the central dogma of molecular biology, which states that proteins are translated from RNA, which is transcribed from DNA. This dogma has since been shown to have exceptions, such as reverse transcription in retroviruses. The modern study of genetics at the level of DNA is known as molecular genetics.
In 1972,
Walter Fiers and his team were the first to determine the sequence of a gene: that of Bacteriophage MS2 coat protein.[18] The subsequent development of chain-termination
DNA sequencing in 1977 by
Frederick Sanger improved the efficiency of sequencing and turned it into a routine laboratory tool.[19] An automated version of the
Sanger method was used in the early phases of the Human Genome Project.[20]
Modern synthesis and its successors
Modern synthesis (20th century)
The theories developed in the early 20th century to integrate Mendelian genetics with Darwinian evolution are called the modern synthesis, a term introduced by Julian Huxley.[21]
Evolutionary biologists have subsequently modified this concept, such as George C. Williams' gene-centric view of evolution. He proposed an evolutionary concept of the gene as a unit of natural selection with the definition: "that which segregates and recombines with appreciable frequency."[22]:24 In this view, the molecular gene transcribes as a unit, and the evolutionary gene inherits as a unit. Related ideas emphasizing the centrality of genes in evolution were popularized by Richard Dawkins.[23][24]
DNA
The vast majority of organisms encode their genes in long strands of DNA (deoxyribonucleic acid). DNA consists of a chain made from four types of nucleotide subunits, each composed of: a
five-carbon sugar (2-deoxyribose), a
phosphate group, and one of the four bases
adenine,
cytosine,
guanine, and
thymine.[25]:2.1
Two chains of DNA twist around each other to form a DNA double helix with the phosphate-sugar backbone spiraling around the outside, and the bases pointing inwards with adenine base pairing to thymine and guanine to cytosine.
The specificity of base pairing occurs because adenine and thymine align to form two hydrogen bonds, whereas cytosine and guanine form three hydrogen bonds. The two strands in a double helix must, therefore, be complementary, with their sequence of bases matching such that the adenines of one strand are paired with the thymines of the other strand, and so on.[25]:4.1
Due to the chemical composition of the pentose residues of the bases, DNA strands have directionality. One end of a DNA polymer contains an exposed hydroxyl group on the deoxyribose; this is known as the 3' end of the molecule.
The other end contains an exposed phosphate group; this is the 5' end. The two strands of a double-helix run in opposite directions. Nucleic acid synthesis, including DNA replication and transcription, occurs in the 5'→3' direction because new nucleotides are added via a dehydration reaction that uses the exposed 3' hydroxyl as a nucleophile.[26]:27.2
The expression of genes encoded in
DNA begins by transcribing the gene into
RNA, the second type of nucleic acid that is very similar to DNA, but whose
monomers contain the sugar ribose rather than deoxyribose. RNA also contains the base uracil in place of thymine. RNA molecules are less stable than DNA and are typically single-stranded.
Genes that encode proteins are composed of a series of three-nucleotide sequences called codons, which serve as the "words" in the genetic "language". The genetic code specifies the correspondence during protein translation between codons and amino acids. The genetic code is nearly the same for all known organisms.[25]:4.1
Chromosomes
A microscopy image of 46 chromosomes striped with red and green bands
Fluorescent microscopy image of a human female karyotype, showing 23 pairs of chromosomes. The DNA is stained red, with regions rich in housekeeping genes further stained in green. The largest chromosomes are around 10 times the size of the smallest.[27]
The total complement of genes in an organism or cell is known as its genome, which may be stored on one or more chromosomes. A chromosome consists of a single, very long DNA helix on which thousands of genes are encoded.[25]:4.2
The region of the chromosome at which a particular gene is located is called its locus. Each locus contains one allele of a gene; however, members of a population may have different alleles at the locus, each with a slightly different gene sequence.
The majority of
eukaryotic genes are stored on a set of large, linear chromosomes. The chromosomes are packed within the nucleus in complex with storage proteins called histones to form a unit called a nucleosome.
DNA packaged and condensed in this way is called
chromatin.[25]:4.2
The manner in which
DNA is stored on the histones, as well as chemical modifications of the histone itself, regulate whether a particular region of DNA is accessible for gene expression. In addition to genes,
eukaryotic chromosomes contain sequences involved in ensuring that the
DNA is copied without degradation of end regions and sorted into daughter cells during cell division:
replication origins,
telomeres, and the
centromere.[25]:4.2
Replication origins are the sequence regions where
DNA replication is initiated to make two copies of the chromosome. Telomeres are long stretches of repetitive sequences that cap the ends of the linear chromosomes and prevent degradation of coding and regulatory regions during
DNA replication.
The length of the telomeres decreases each time the genome is replicated and has been implicated in the aging process.[28] The centromere is required for binding spindle fibers to separate sister chromatids into daughter cells during cell division.[25]:18.2
Prokaryotes (bacteria and archaea) typically store their genomes on a single large, circular chromosome. Similarly, some eukaryotic organelles contain a remnant circular chromosome with a small number of genes.[25]:14.4
Prokaryotes sometimes supplement their chromosome with additional small circles of
DNA called plasmids, which usually encode only a few genes and are transferable between individuals. For example, the genes for antibiotic resistance are usually encoded on bacterial plasmids and can be passed between individual cells, even those of different species, via horizontal gene transfer.[29]
Whereas the chromosomes of
prokaryotes are relatively gene-dense, those of
eukaryotes often contain regions of
DNA that serve no obvious function. Simple single-celled eukaryotes have relatively small amounts of such
DNA, whereas the genomes of complex multicellular organisms, including humans, contain an absolute majority of DNA without an identified function.[30]
This
DNA has often been referred to as "junk DNA". However, more recent analyses suggest that, although protein-coding DNA makes up barely 2% of the human genome, about 80% of the bases in the genome may be expressed, so the term "junk DNA" may be a misnomer.[4]
Structure and function Structure
The structure of a gene consists of many elements of which the actual protein-coding sequence is often only a small part. These include DNA regions that are not transcribed as well as untranslated regions of the RNA.
Flanking the open reading frame, genes contain a regulatory sequence that is required for their expression. First, genes require a promoter sequence. The promoter is recognized and bound by transcription factors that recruit and help RNA polymerase bind to the region to initiate transcription.[25]:7.1 The recognition typically occurs as a consensus sequence like the
TATA box.
A gene can have more than one promoter, resulting in messenger RNAs (mRNA) that differ in how far they extend in the 5' end.[32] Highly transcribed genes have "strong" promoter sequences that form strong associations with transcription factors, thereby initiating transcription at a high rate. Other genes have "weak" promoters that form weak associations with transcription factors and initiate transcription less frequently.[25]:7.2
Eukaryotic promoter regions are much more complex and difficult to identify than prokaryotic promoters.[25]:7.3
Additionally, genes can have regulatory regions many
kilobases upstream or downstream of the open reading frame that alters expression. These act by binding to transcription factors which then cause the DNA to loop so that the regulatory sequence (and bound transcription factor) become close to the RNA polymerase binding site.[33] For example, enhancers increase transcription by binding an activator protein which then helps to recruit the RNA polymerase to the promoter; conversely, silencers bind repressor proteins and make the DNA less available for RNA polymerase.[34]
The transcribed
pre-mRNA contains untranslated regions at both ends which contain a ribosome binding site, terminator and start and stop codons.[35] In addition, most eukaryotic open reading frames contain untranslated introns which are removed before the exons are translated. The sequences at the ends of the introns dictate the splice sites to generate the final mature mRNA which encodes the protein or
RNA product.[36]
Many prokaryotic genes are organized into operons, with multiple protein-coding sequences that are transcribed as a unit.[37][38] The genes in an operon are transcribed as a continuous messenger RNA, referred to as a polycistronic mRNA. The term
cistron in this context is equivalent to genes. The transcription of an
operon's mRNA is often controlled by a repressor that can occur in an active or inactive state depending on the presence of specific metabolites.[39]
When active, the repressor binds to a DNA sequence at the beginning of the operon, called the operator region, and represses transcription of the operon; when the repressor is an inactive transcription of the operon can occur (see e.g. Lac operon). The products of operon genes typically have related functions and are involved in the same regulatory network.[25]:7.3
https://en.wikipedia.org/wiki/Gene
Deoxyribonucleic acid (/diːˈɒksɪˌraɪboʊnjuːˌkliːɪk, -ˌkleɪ-/ (About this sound listen);[1]
DNA) is a molecule composed of two polynucleotide chains that coil around each other to form a double helix carrying genetic instructions for the development, functioning, growth, and reproduction of all known organisms and many viruses. DNA and ribonucleic acid (RNA) are nucleic acids. Alongside proteins, lipids and complex carbohydrates (polysaccharides), nucleic acids are one of the four major types of macromolecules that are essential for all known forms of life.
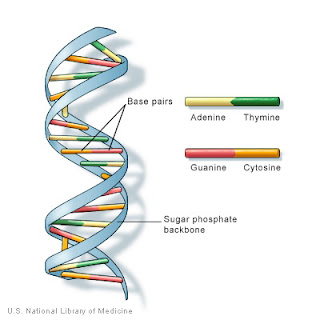
The two DNA strands are known as polynucleotides as they are composed of simpler monomeric units called nucleotides.[2][3] Each nucleotide is composed of one of four nitrogen-containing nucleobases (cytosine [C], guanine [G], adenine [A] or thymine [T]), a sugar called deoxyribose, and a phosphate group. The nucleotides are joined to one another in a chain by covalent bonds (known as the phospho-diester linkage) between the sugar of one nucleotide and the phosphate of the next, resulting in an alternating sugar-phosphate backbone.
The nitrogenous bases of the two separate polynucleotide strands are bound together, according to base-pairing rules (A with T and C with G), with hydrogen bonds to make double-stranded DNA. The complementary nitrogenous bases are divided into two groups, pyrimidines, and purines. In DNA, the pyrimidines are thymine and cytosine; the purines are adenine and guanine.
Ribonucleic acid (RNA) is a polymeric molecule essential in various biological roles in coding, decoding, regulation, and expression of genes. RNA and DNA are nucleic acids, and, along with lipids, proteins and carbohydrates, constitute the four major macromolecules essential for all known forms of life. Like DNA, RNA is assembled as a chain of nucleotides, but unlike DNA, RNA is found in nature as a single-strand folded onto itself, rather than a paired double-strand.
Cellular organisms use messenger RNA (mRNA) to convey genetic information (using the nitrogenous bases of
guanine,
uracil,
adenine, and
cytosine, denoted by the letters G, U, A, and C) that directs the synthesis of specific proteins. Many viruses encode their genetic information using an RNA genome.
Some RNA molecules play an active role within cells by catalyzing biological reactions, controlling gene expression, or sensing and communicating responses to cellular signals. One of these active processes is protein synthesis, a universal function in which RNA molecules direct the synthesis of proteins on ribosomes.
This process uses transfer RNA (tRNA) molecules to deliver amino acids to the ribosome, where ribosomal RNA (rRNA) then links amino acids together to form coded proteins.
Reference Link
https://en.wikipedia.org/wiki/RNA
Sources
Main textbook
Alberts B, Johnson A, Lewis J, Raff M, Roberts K, Walter P (2002). Molecular Biology of the Cell (Fourth ed.). New York: Garland Science. ISBN 978-0-8153-3218-3. – A molecular biology textbook available free online through NCBI Bookshelf